An interview with Dr. Sara Mahshid
By determining the order of the nucleotides in several DNA strands, DNA sequencing helps understanding genetic mechanisms. The ability to see where a specific gene is offers a new way for the treatment of genetic diseases: regulatory instructions in the DNA can be altered by adding genes or by turning genes off. Since many biological fields are expanding, researchers need automated and reliable methods to enhance the experiments. I had the pleasure of sitting down with Dr. Sara Mahshid to discuss about a new device that allows the fast sequencing of DNA and could help identifying a new cancer marker. She recently published a paper in Scientific Reports describing this new method applied to DNA linearization. If this technique were to work effectively, researchers would have their DNA sequencing projects carried out more efficiently and reliably.
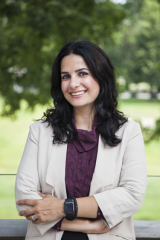
Mahshid Research Group
Dr. Sara Mahshid graduated from Sharif University of Technology and has a PhD in Materials Engineering applied on the development of a biosensor based on titanium oxide nanotubes. She started her lab in August 2017 in the Department of Bioengineering at McGill. The research group actively began its projects recently, in winter 2018, when her graduate students joined.
Their research is based on a new approach in nanofluidic sensors, using devices which integrate nanosurfaces and nanopatterned platforms. The field of application for this device is quite broad, it could help with drug discovery, DNA sequencing, cell growth, cell differentiation, biosensing and bacteria detection.
To conduct these projects, her team is composed of both biologists and engineers from different disciplines such as materials, mechanical, and electrical engineering.
DNA linearization project
Although DNA sequencing is already routinely performed with well-known methods, there remains a high demand for devices allowing on-chip loading and linearizing DNA that would avoid the molecule’s fragmentation. The figure 1 below shows the difference of observation between unstretched and linearized DNA on a biochip. One big advantage of biochips is to visualize all the DNA molecules in one field of view. These approaches should be simple, reliable and cost-effective. The goal of this project was to develop a solution to confine and stretch DNA molecules to directly observe them within a single field of view in order to acquire contextual information about the sequences of DNA. Therefore, it could help mapping DNA by matching different sequences of DNA and their location in the DNA strand.
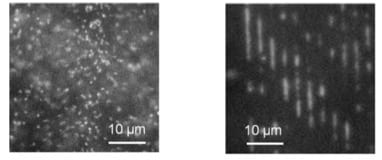
– unstretched DNA molecules on the platform (left).
– linearized DNA molecules in nanocavities (right).
DNA has the interesting ability of confining inside a nanofluidic channel. Because of its attraction to the channel wall, it can readily linearize, thereby simplifying the DNA sequencing process. To read the sequence of the DNA, different approaches using fluidic channels are being developed to look at the DNA base pairs inside a confined area. They can be categorized into three approaches: fixed confinement, which uses a hydrodynamic loading; tuneable confinement, which uses mechanical force todeform a membrane; and free surface stretching, where DNA is stretched on a free surface with an optical, magnetic, or electric field.
During her post-doc, Sara Mahshid worked on standard nanoconfinement technologies using hydraulic force. Despite their advantages, she noticed several shortcomings such as low throughput leading to time-consuming technical processes and risk of DNA fragmentation, leading to loss of information. Indeed, one of the challenges is that these methods are limited by a very high hydraulic resistance between the microscale inlet and the nanofluidic region. With hydrodynamic forces to load DNA from a side channel, a high pressure is needed to overcome the hydraulic resistance, which can result in DNA fragmentation. While one would like to decrease the channel size to visualize DNA, the smaller the channel size, the more difficult it is to load DNA inside it with this technique.
Thus, she was looking for a method that could lead to a high throughput without fragmenting the DNA. This is how this project started: instead of loading the DNA from the side channel to the fluidic channel, she developed a system which would load DNA from the top thanks to the action of an electric field.
How does it work?
As a matter of facts, in these experiments, the DNA molecules can be treated as dielectric particles because they can sense electric fields. Dielectrophoresis (DEP) force fields load DNA inside the channel and confine it in the nanocavities, as showed in the figure 2. Moreover, DEP force is easily controlled by modifying the frequency of the electric field. In this way, there is no need for bonding, hydrodynamic nor mechanical forces anymore, preventing DNA molecules from fragmenting. Furthermore, because they load the DNA from top, they do not need applying any external forces.
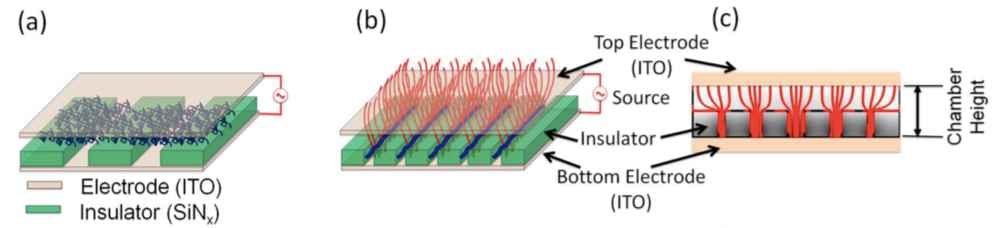
They developed a numerical simulation with 2D finite elements to adapt the design of the platform by tracking particle trajectory variations as a function of the frequency of the electric current. They observed that DNA molecules did not load into the nanogrooves at low frequency, but they could establish a frequency threshold (100 kHz) where DNA would tend to concentrate at the bottom of the cavities. This simulation allowed them to optimize the size of the electrodes. To prove the concept described by their numerical simulation, they conducted several experiments. So far in their tests, the DEP force has not been high enough to fragment small strands of DNA (about 40kpb), though Dr. Mahshid mentions that they have yet to try their platform on longer strands such as human genomic DNA. You can see in the figure 3 an example of DNA molecules concentrating along an electrode when DEP force is applied.
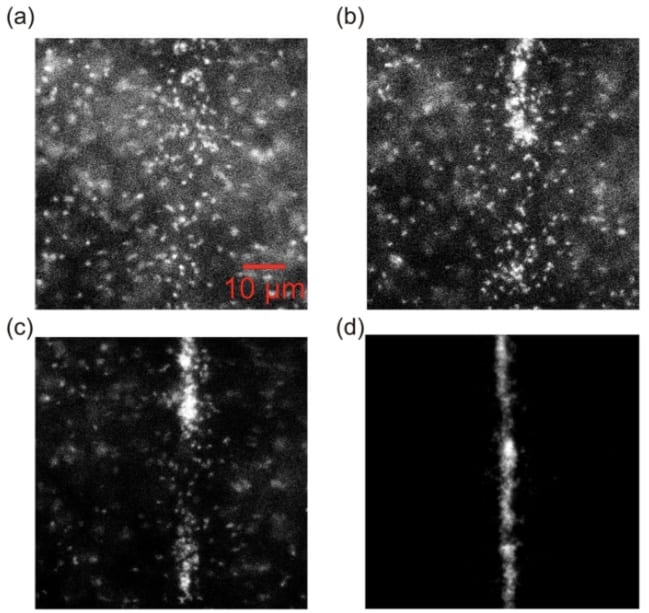
(a) DNA in free solution.
(b) Application of DEP force, DNA molecules start to move towards the electrode (which is a vertical rectangle in the center of the image).
(c, d) DNA molecules concentrate along the electrode.
Once the DEP field is generated, the loading is very fast. The DNA will sense the field and move in the desired direction (positive or negative depending on the DEP field polarity) before becoming trapped within a nanogroove. The speed depends on the size of the particle, and the strength of the field: the stronger the field is, and the smaller the particle is, the faster the motion will be. As long as the field is applied and the array of nanofeatures is in the field of view of the microscope, it is possible to see the DNA directly trapped inside the nanocavities. Conversely, it is possible to unload DNA from the nanofeatures by switching the DEP effect. Therefore, it is easy to have access to contextual information of DNA sequences in one set of images. Since they use nanofabrication techniques to pattern their nanoplatform in parallel arrays of nanochannel and nanocavities, the size of this array can be adjusted to the size of the microscope’s field of view.
Micro and nano-fabrication techniques used for this device are standard techniques based on electron beam lithography (a very expensive fabrication method) and UV lithography. These methods of lithography consist in giving a pattern to a photosensitive or electron-sensitive film, called a “resist”. First, the glass platform is entirely covered by the resist, after which the selected parts of the resist have their solubility changed by UV light or e-beam, depending on the application. Following this, the resist is immersed in a solvent to remove the soluble parts: this process is called developing. When the resist is developed, the team etch the substrate to transfer the features and the patterns from the resist to the nanoplatform. The non-soluble remaining resist protects the platform from being etched in the chosen areas. At the end of the fabrication, Sara Mahshid’s team characterized the surface of their devices with Atomic Force Microscopy (AFM) scans to ensure the surface was smooth enough so that the DNA will not stick to the channels. One of the directions of research in this laboratory is to look for more cost-effective fabrication protocols such as direct writing.
On-going research
Dr Mahsid’s team is currently using DEP and nanoplatforms for different types of applications such as confinement, separation and sorting of particles. The DEP force is very much dependent on the geometry and size of the electrodes. So, the investigations in each project start by finding a suitable geometry for the current application. One of their current nanopatterned projects is to target exosomes and confine them with DEP. They hope they can isolate exosomes based on their single-level technique using a nanopatterned platform.
The medical application of confining exosomes is for diagnosis, as exosomes are a cancer marker. Such an advance for confining and trapping exosomes in nanocavities would help analyzing their molecular content with in situ analyzing and extraction of DNA. This device would help identifying the cancer type and would enable clinicians to customize the treatment for each patient. The experiments of Sara Mahshid’s research group have been encouraging so far. They were able to capture and confine exosomes in nanocavities, when working with pure exosomes brought from culture. As for clinical samples, they first need to purify them before confining the exosomes.
In conclusion, the nanoplatform combined with DEP is pretty much a universal method that can be used for cells, DNA, exosomes and other types of particles. Theoretically it only needs the particle to react as a dielectric particle once the platform’s features are adapted to the particles’ size. It could then revolutionize the current confinement method for small particles.
Visit Mahshid research group for more information about their lab and research.
_______________________________________________________________________________________________________________
Reference:
Mahshid, Sara & Lu, Jia & A. Abidi, Abrar & Sladek, Robert & W. Reisner, Walter & Ahamed, Mohammed. (2018). Transverse dielectrophoretic-based DNA nanoscale confinement. Scientific Reports.8. 10.1038/s41598-018-24132-5